I always wanted to understand life. What moves us? What allows us to heal and thrive? And what goes wrong when we get sick or when we eventually stop breathing and die? My search for answers to these stupendously ambitious questions led me, it now seems inexorably, to mitochondria.
In biology classes from high school through university, I learned that mitochondria are little objects that reside within each cell and serve as “powerhouses,” combining oxygen and food to yield energy for the body. This idea of mitochondria being little batteries with a built-in charger, about as interesting as the one in my phone, left me unprepared for the vital reality of these organelles when I first saw them under a microscope in 2011. They were luminous because of a glowing dye I had put in them, and they were dynamic—constantly moving, stretching, morphing, touching one another. They were beautiful. That night, a graduate student alone in a dark laboratory in Newcastle upon Tyne in England, I became a mitochondriac: hooked on mitochondria.
A profound insight by biologist Lynn Margulis helped me make some sense of what I was seeing. She postulated in 1967 that mitochondria descend from a bacterium that was engulfed by a larger ancestral cell about 1.5 billion years ago. Instead of consuming this tidbit, the larger cell let it continue living within. Margulis called this event endosymbiosis, which means, roughly, “living or working together from the inside.” The host cell had no energy source that used oxygen—which, thanks to plants, was already abundant in the atmosphere; mitochondria filled this gap. The unlikely union allowed cells to communicate and cooperate and let their awareness expand beyond their own boundaries, enabling a more complex future in the form of multicellular animals. Mitochondria made cells social, binding them in a contract whereby the survival of each cell depends on every other one, and thus made us possible.
On supporting science journalism
If you're enjoying this article, consider supporting our award-winning journalism by subscribing. By purchasing a subscription you are helping to ensure the future of impactful stories about the discoveries and ideas shaping our world today.
Amazingly, my co-workers and I have discovered that mitochondria are themselves social beings. At least, they foreshadow sociality. Like the bacterium they descended from, they have a life cycle: old ones die out, and new ones are born out of existing ones. Communities of these organelles live within each cell, usually clustered around the nucleus. Mitochondria communicate, both within their own cells and among other cells, reaching out to support one another in times of need and generally helping the community flourish. They produce the heat that keeps our bodies warm. They receive signals about aspects of the environment in which we live, such as air pollution levels and stress triggers, and then integrate this information and emit signals such as molecules that regulate processes within the cell and, indeed, throughout the body.
When our mitochondria thrive, so do we. When they malfunction—when, for instance, their ability to change energy into forms required for biochemical reactions is impaired—we may experience conditions as diverse as diabetes, cancer, autism and neurodegenerative disorders. And as mitochondria accumulate defects over a lifetime of stress and other insults, they contribute to aging and, ultimately, death. To understand these processes—to see how to sustain physical and mental health—it helps to understand how energy moves through our bodies and minds. That requires a deeper look into mitochondria and their social lives.
Long before I got my first glimpse of mitochondria, I had boned up on the basics of their structure and biology. We inherit our mitochondria from our mother—from the egg cell, to be precise. Mitochondria have their own DNA, which consists of only 37 genes, compared with the thousands of genes in the spiraling chromosomes inside the cell nucleus. This ring of mitochondrial DNA, or mtDNA, is sheltered within two membranes. The outer shell, shaped like the skin of a sausage, encases the mitochondrion and selectively allows molecules to enter or exit. The inner membrane is made of densely packed proteins and has many folds, called cristae, which serve as a site for chemical reactions, much like the plates suspended inside a battery.
Rather than being like battery chargers, mitochondria are more like the motherboard of the cell.
In the 1960s British biochemists Peter Mitchell and Jennifer Moyle discovered how electrons derived from carbon in food combine with oxygen in the cristae, releasing a spark of energy that is captured as a gradient in electrical voltage across the membrane. This voltage provides the driving force for all processes in the body and brain, from warming to manufacturing molecules to thinking. Mitochondria also produce a molecule called adenosine triphosphate, which serves as a portable unit of energy that powers hundreds of biochemical reactions within each cell.
Returning from the U.K., I started a postdoctoral fellowship with geneticist and evolutionary biologist Douglas Wallace at the Center for Mitochondrial and Epigenomic Medicine at Children’s Hospital of Philadelphia. In 1988 Wallace had discovered the first-ever link between a mutation in mtDNA and a human disease. He had gone on to map some of mitochondrial biology’s fundamental connections to various diseases and the aging process, laying the foundation of the field of mitochondrial medicine. In Philadelphia, I began working with a fellow postdoc, Meagan McManus, who wanted to understand how defective mitochondria could cause cardiovascular and neurological diseases. McManus asked me to photograph with an electron microscope the mitochondria in the hearts of mice with a specific mtDNA mutation that led to heart failure.
Our team was also experimenting with three-dimensional imaging using electron tomography, the same technology that allows a radiologist to see a patient’s internal organs in 3D. Weeks later, the director of this project, Dewight Williams of the University of Pennsylvania, brought me to a room where the million-dollar tomography microscope stood, as high as the ceiling, to show me reconstructed movies of mitochondria.
The tomography gave us a 3D view of the cristae. Some mitochondria in the hearts of the sick mice had jagged, highly irregular cristae—the unhealthy look I had been seeing in the 2D pictures. But one thing showed up in 3D that we had never seen in the flat images: even when mitochondria looked unhealthy, their cristae looked healthy at places where the mitochondria touched one another. They were interacting, helping one another’s internal organization. These mito-mito junctions also had more cristae than any other part of the same mitochondrion. “Meagan has to see this!” I thought, rushing to the lab across campus.
As I restarted the movie for McManus, I narrated what I had seen a few minutes earlier: “Mitochondria are influencing one another!” We watched the looping video a few times. Then McManus said, her voice pitched high with excitement, “And the cristae line up! The cristae line up between mitochondria!” She drew a line with her extended finger across a junction between mitochondria.
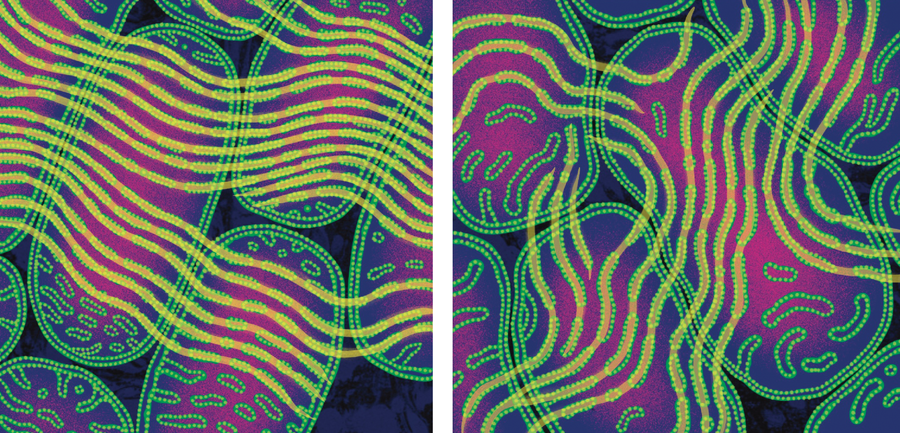
Jennifer N. R. Smith
I had pored over thousands of electron microscopy images from the best microscopists. Never had I heard about cristae in one mitochondrion aligning with the cristae of another mitochondrion. While in Newcastle, I had seen a 1983 paper by Russian scientists Lora E. Bakeeva and Vladimir P. Skulachev describing “intermitochondrial contacts,” and I had demonstrated that these contacts increased after exercise—perhaps increasing energy efficiency. How had we all missed the alignment? Yet instead of lying there as parallel plates, like textbooks often portrayed them, the cristae formed parallel ribbons undulating across mitochondria. It almost looked like the cristae were helping their neighbors organize to achieve the typical, healthy, regular array.
At the next lab meeting, I suggested that these patterns looked like iron filings aligned around a magnet. Cristae are full of iron-sulfur clusters that may be paramagnetic. If they are, maybe there were electromagnetic fields induced by the flow of electrical charge across the cristae? Could they induce the cristae to line up? So far this hypothesis appears to be the best one for how cristae align across mitochondria. For me, it also opened the door to thinking about how the forces of physics might have contributed to the evolution of multicellular life—all the way to us.
This discovery and the thoughts it spurred changed my view of mitochondria forever. Hundreds of hours in the dark dungeon where I studied mitochondria and numerous collaborations later, I had learned one important lesson: mitochondria exchange information. The fingerprint of that exchange lay right there in the patterns of their cristae. Further studies at the University of Tsukuba in Japan and elsewhere, using cells with varying levels of mitochondrial dysfunction caused by mtDNA mutations, showed that healthy mitochondria can donate intact mtDNA to mutant mitochondria. In conditions of scarce energy supply, mitochondria fuse with one another into long strands to share mtDNA. Isolated mitochondria without mtDNA or with mutated mtDNA can similarly fuse with healthy mitochondria, restoring their normal function.
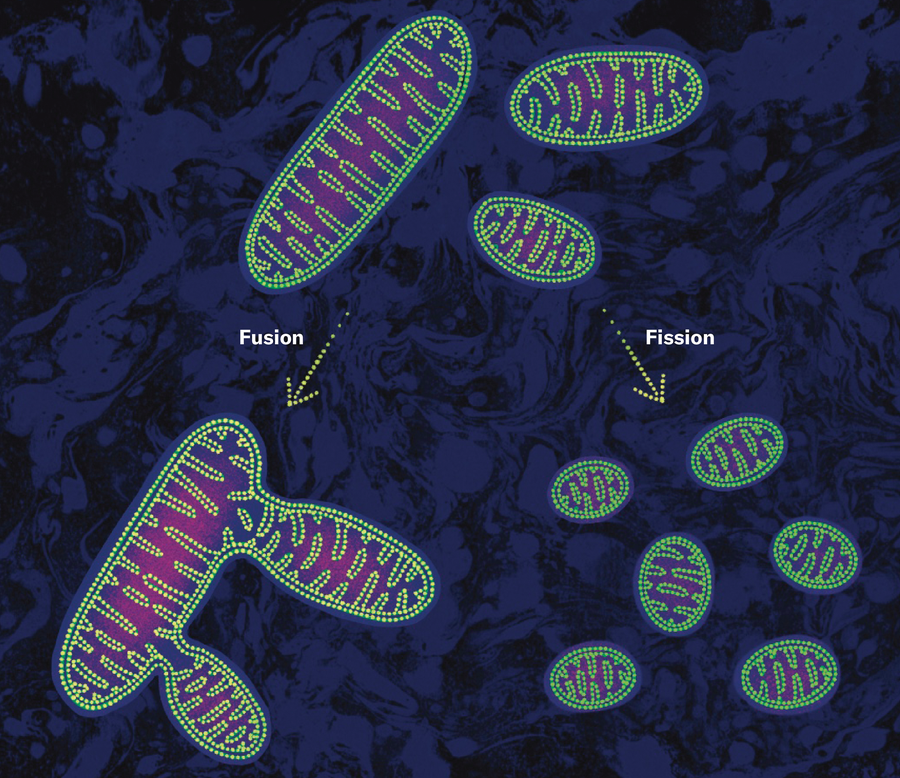
Jennifer N. R. Smith
Fusion enhances the resilience not only of mitochondria but also of cells; interfering with these interactions leads to isolated mitochondria that accumulate mtDNA defects and ultimately die, along with the cells they live in. In people, decreased levels of mitofusin 2, a protein located in the outer mitochondrial membrane that helps with fusion, are correlated with neurodegeneration. And mice with mitochondria that have been engineered to impede fusion in the nucleus accumbens, a brain region involved in regulating reward, are more anxious.
Could there be yet other ways in which mitochondria communicate? Could they act like their bacterial ancestors, which build biofilms and use membrane protrusions, electrical fields and secreted molecules to cooperate and conquer the living world with their versatile collective behaviors? Could mitochondrial communication reveal a broader internal universe of energy and information exchange? Could mitochondrial junctions and aligned cristae operate like neuronal synapses, with the resulting mitochondrial collective behaving essentially like an intracellular brain?
In 2016, shortly after starting my own lab at Columbia University, I was back in Newcastle on a visit to Doug Turnbull’s Wellcome Center for Mitochondrial Research. I was again sitting at the electron microscope, this time with a stellar British graduate student, Amy Vincent. We were imaging muscle from the calf of a woman with an mtDNA mutation that caused a rare mitochondrial disease. By coincidence, her mutation was similar to the one McManus’s mice had had.
What Vincent and I found that afternoon opened another avenue of inquiry. In front of our eyes lay mitochondrial nanotunnels: thin membrane protrusions—the same kind that bacteria use to share their circular DNA! For the first time in humans, Vincent and I saw that mitochondria send thin tubular structures out toward one another, like feelers that some solitary cells use to search for a more hospitable environment or a healthy fellow cell. By imaging dozens of other muscle samples, we found that people whose mitochondria don’t work well have more nanotunnels. It was as if unhealthy mitochondria with mtDNA mutations were reaching out for help.
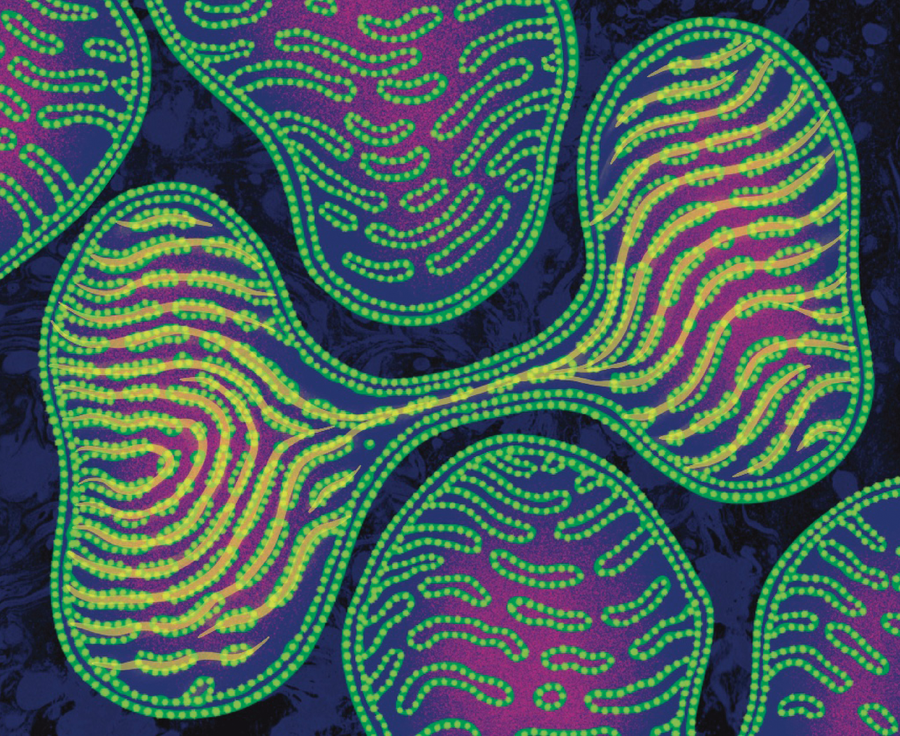
Jennifer N. R. Smith
Perhaps the most remarkable aspect of the mitochondrial collective, however, is that mitochondria from different parts of the body talk to one another, using hormones as their language. Mitochondria produce the steroid hormones we use for sustaining and reproducing life. Cortisol, the hormone that increases blood glucose levels to fuel the stress response, is made in the mitochondria of the adrenal glands, which sit on top of the kidneys. Testosterone, estrogen and progesterone are synthesized mainly by mitochondria in the reproductive organs. Interestingly, brain mitochondria have receptors to sense both stress and sex hormones. So we have a population of mitochondria in the adrenal glands that signal directly, via the blood, to mitochondria in the brain.
Further, mitochondria are not all created equal. In the same way that humans develop specialties in different social and economic roles and organs specialize in executing complementary functions (the liver feeds other organs, the heart pumps, the brain integrates information and issues directives), mitochondria also specialize. Across organs and cell types, mitochondria look different. Their protein contents are different. They move differently. And their ability to sense, integrate and signal specific information varies according to the cell they inhabit. Mitochondrial specialization most likely affords gains in efficiency, allowing an organism to survive at a lower overall energy cost.
My co-workers and I recently built the first map of mitochondria in the human brain. Even within this single organ there are different types of mitochondria in different parts of the cortex and in deeper, subcortical brain regions. The brain uses 20 percent of the body’s energy, despite constituting only 2 percent of the body’s mass, so an efficient source of power is critical to its functioning. My colleagues, notably, Michel Thiebaut de Schotten of the French National Center for Scientific Research and Eugene V. Mosharov of Columbia, and I found that the more recently evolved brain areas, which have the highest energy expenditure, have mitochondria that are more strongly specialized for energy transformation.
Mitochondria within a cell may also look very different from one another. For example, in neurons, “dendritic” mitochondria are found in the fibers, or dendrites, through which neurons receive signals from other cells. These mitochondria are stable filaments that stretch over 10 to 30 microns—a stupendously long distance for this type of structure—and have several mtDNA copies. “Axonal” mitochondria move along the linear axons, which conduct signals to other neurons, as if they were cellular highways. They are generally short and stubby (up to a micron in length), and many lack mtDNA. “Cytoplasmic” mitochondria cluster around the nucleus and look like something between the dendritic and axonal types. Similar grouping and specialization of mitochondria exists in muscle and fat cells.
These findings, taken together, led behavioral neuroscientist Carmen Sandi of the Swiss Federal Institute of Technology and me to propose in 2021 that mitochondria are social organelles. If you are like me and your eyebrow rises when you hear the term “social” applied to a subcellular organelle, you are having a normal reflex. Nevertheless, Sandi and I argue that mitochondria show all the features of social beings—a shared environment inside the cell or body, communication, formation of groups or types, synchronization of behavior, interdependence, and specialization in the tasks they perform.
In a subsequent paper, which required a painfully long review of more than 400 studies, Orian S. Shirihai of the University of California, Los Angeles, and I established that the mitochondrial collective operates as a mitochondrial information-processing system, or MIPS. Like the animals they exist within and support, which must respond flexibly to the environment, the mitochondria sense signals, integrate this information in the membrane potential of their cristae, and produce signals that regulate the genes of the cell and shape cell behavior.
Your eyes transform light into electrical impulses that coalesce into an image in your visual field, and your ears transform air-pressure waves into electrical pulses that you eventually perceive as sounds. Likewise, mitochondria transform dozens of hormonal, metabolic, chemical, and other information streams into their electrical membrane potential. This “bioenergetic” state then leads to the production of secondary messenger molecules that are intelligible to the nucleus. So in the same way you read messages on your phone, which receives signals, transforms them and projects decipherable information onto its screen, the nucleus of your cells can “read” the environment through the MIPS that surrounds it.
Rather than having supplementary roles like those of battery chargers, mitochondria are more like the motherboard of the cell. Genes sit inert in the nucleus until energy and the right message come along to turn some of them on and some others off. Mitochondria provide these messages, speaking the language of the epigenome—the malleable layer of regulation that sits on top of the genome to regulate its expression.
My colleague Timothy Shutt of the University of Calgary likes to call mitochondria the “CEO of the cell”: the chief executive organelle. This metaphor captures how mitochondria not only are involved in integrating information but also give orders. They dictate whether the cell divides, differentiates or dies. Indeed, mitochondria have a veto on cell life or death. If the MIPS deems it necessary, it triggers programmed cell death, or apoptosis—a form of self-sacrifice for the greater good of the organism.
So vital are mitochondria that in difficult times cells may donate entire mitochondria to other cells. “In cellular emergencies, newly arrived mitochondria might kick-start tissue repair, fire up the immune system or rescue distressed cells from death,” journalist Gemma Conroy noted in a Nature news story last April. Inside tumors, cancer cells and immune cells appear to compete for mitochondria, using them as a kind of bioweapon. An international effort I participated in, led by Jonathan R. Brestoff of the Washington University School of Medicine in St. Louis, recently created an entirely new lexicon to guide the emerging field of mitochondria transfer and transplantation.
All well and good, you may think. What does all this mean for my health or how long I’m going to live?
The short answer is that it may have everything to do with human health. Diabetes, neurodegenerative conditions, cancer and even mental health illnesses are all emerging as metabolic disorders involving malfunctioning mitochondria. And these findings are indicating new routes for intervention.
Mitochondria drive health—or disease—in several ways. One route derives from their role as energy processors. In an electrical circuit, if we crank up the input voltage too much, we can blow it out. Similarly, if our cells are exposed to too much glucose or fat—or, worse, both together, causing what doctors refer to as glucolipotoxicity—the mitochondria undergo fission and fragment into little bits, accumulate mtDNA defects, and produce signals that end up prematurely aging or killing the cell. Experiments in cells and in mice have shown that pharmacologically or genetically preventing mitochondrial fission induced by excessive glucose and fats may protect against insulin resistance.
Cancer, too, may be a disorder of cellular metabolism. Cancer cells can burn glucose without oxygen, which suggests either that something is wrong with their mitochondria or that they prefer to reserve mitochondria for use in cell division—and proliferation.
A second pathway is through mitochondria’s influence on gene expression. Mitochondrial signals alter the expression of more than 66 percent of genes in the nuclear chromosomes. By changing which genes are expressed and to what extent, mutations in mtDNA may completely alter the nature, behavior and stress resilience of cells and ultimately of the whole organism.
Mitochondria can look terribly odd when sick. In people with mtDNA defects that cause rare mitochondrial diseases, such as the person in whose mitochondria we first saw nanotunnels, the cristae in particular can look somewhat alien—like crop circles with regular angles, paracrystalline inclusions, and other weird shapes.
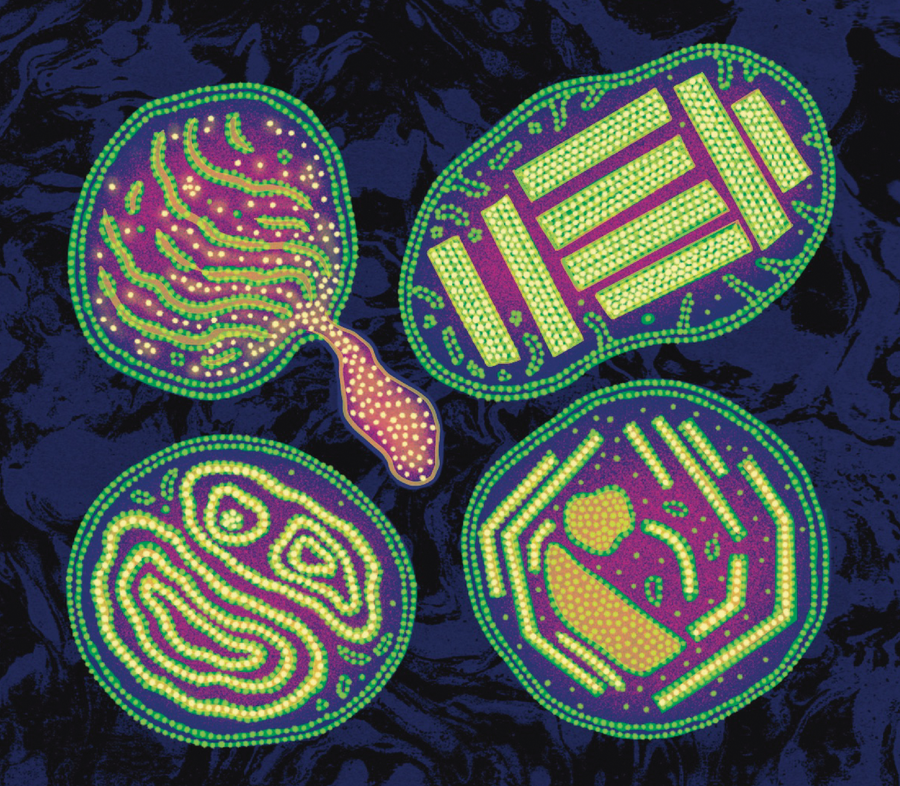
Jennifer N. R. Smith
Notably, abnormal mitochondrial shape and function are emerging as biomarkers and potential causes of cognitive and neurodegenerative disorders such as Alzheimer’s disease, Parkinson’s disease, and others. Clinically, a neurobiological subtype of autism spectrum disorder involves defects in mitochondrial biology.
A third pathway is inflammation. When cells are injured or stressed, they may leak mtDNA into the cell’s interior, the cytoplasm, or even into the blood. Along with Caroline Trumpff of Columbia, Anna Marsland and Brett Kaufman of the University of Pittsburgh, and other co-workers, I found that mental stress induced by having to speak in public for five minutes increased the amount of free-floating mtDNA in the blood. People in intensive care units who are grievously ill tend to have very high levels of mtDNA in their blood. Because mtDNA rings resemble bacterial DNA, immune cells see them as pathogens and mount an attack that can develop into inflammation. And inflammation, clinicians know well, is linked to the onset and progression of a host of chronic health conditions.
Just how defective mitochondria lead to illness in the body and mind is a question that has yet to be answered. But there are simple ways to ensure our mitochondria stay healthy. One is exercise. When you move vigorously, your cells consume energy rapidly, powering up the membrane potential of your mitochondria. If your exercise leaves you feeling out of breath, it is a sign that your mitochondria are working hard. Because the brain-body entity is expert at anticipating and preparing for the future, if you move in a way that activates your mitochondria, your body thinks, “Next time this happens, I’ll be ready!” To get ready, it makes more mitochondria and keeps them working at their best.
Surprisingly, social connections, too, may promote the health of our brain mitochondria. In a major study led by David A. Bennett of Rush Medical College in Chicago, researchers asked hundreds of individuals aged 65 and older in the Chicago area to fill out surveys, take cognitive tests and give blood every year until they died. After death their brains were collected to enable analysis of their mitochondria. My colleague Trumpff used those data to ask whether positive mental states such as feeling purpose in life, optimism and a sense of connectedness—or, in contrast, negative mental states such as perceived stress, depression and social isolation—could be related to the mitochondria’s ability to transform energy.
What Trumpff learned was remarkable: the amount of energy-transforming proteins in mitochondria in the prefrontal cortex was significantly correlated with how many positive and negative experiences people reported in the year before they died. This finding aligned with previous studies relating early-life adversity or daily mood markers such as feelings of love, closeness or trust to mitochondria in blood immune cells. Our states of mind might affect the biology of our mitochondria, modulating how well they transform energy.
Another intervention that can be remarkably effective is diet. Medical ketogenic therapy or “nutritional ketosis,” which involves cutting out all refined sugars, limiting intake of carbohydrates, and making up the calorie difference with more proteins and fats, has been shown to sustainably reverse insulin resistance and type 2 diabetes. The ketogenic diet has been used for decades to ward off seizures and thereby “stabilize” the brain in children and adults with intractable and otherwise incurable epilepsy. A ketogenic diet can even ameliorate the mental state and cognitive function of people with Alzheimer’s. It enhances brain-network stability, a marker of brain aging—and this function may explain why some people on the diet sleep better.
The ketogenic diet can have astonishing effects in other diseases as well, as evinced by the story of Lauren Kennedy West, a Canadian woman diagnosed with schizophrenia and bipolar disease at the age of 25. Her life progressively felt too difficult to navigate, “like there was no space for me in the world,” she explained in a moving account of her journey posted on YouTube last year. In December 2023 West began nutritional ketogenic therapy. A couple of weeks later, she noticed she had more energy. Many of her symptoms lessened. After about nine months she was symptom-free, had tapered her medication in collaboration with her care team, and continued to feel better. Late in 2024 she took her last dose of antipsychotic medication.
West’s experience parallels initial positive results from a pilot trial of 21 people with bipolar disease and schizophrenia. Numerous other clinical trials of the ketogenic diet for people with severe mental illnesses such as schizophrenia, depression, anxiety and obsessive compulsive disorder are currently in progress around the world. (Many of these trials are by funded by the Baszucki Group, a philanthropic foundation formed after Matt Baszucki, the son of the group’s founders, successfully treated his bipolar disease with the ketogenic diet. In 2024 I received the Baszucki Prize in Science, which helps to fund my lab at Columbia.)
A new study of 28,995 people in the U.S., 4,484 of whom had significant depression symptoms, also supports the protective effects of low-sugar diets on mental health. People whose diet was “more ketogenic”—low in carbohydrates and sugars relative to lipids and proteins—were less than half as likely to develop depression compared with people whose diet was fairly rich in sugars.
How does it work? From a mitocentric perspective, the ketogenic diet does three things. First, it leads to the supply of an efficient fuel source by the liver, which feeds other organs in the body. If you fast or eat a ketogenic diet, your liver takes the fats from your love handles or your food and breaks them down into smaller bits called ketone bodies. This process happens inside the liver’s mitochondria. Second, after entering the blood, ketone bodies reach the organs, some of which, including the brain, prefer ketone bodies over other fuels such as glucose, proteins and fats. So in the presence of various fuel sources, the brain will preferentially burn ketones.
The third thing may have to do with efficiency—and might explain why ketone bodies are the preferred fuel for the brain. Glucose has to traverse a number of hurdles before getting to neuron mitochondria—it detours through astrocytes, crosses several membranes and goes through several enzymatic reactions. In contrast, ketone bodies are taken up directly by the mitochondria in neurons, where they are burned. It’s a far less convoluted path.
So ketosis, or the burning of ketone bodies, may exert its effects on the brain by enabling energy to directly flow between mitochondria. Ketones in your blood open a stream of communication between producer and consumer mitochondria, fostering their sociality throughout your body.
Once we regard mitochondria as dynamic energy and information processors, an entirely new perspective of life emerges. Think of yourself as a waterfall. The waterfall exists only insofar as the water molecules keep flowing down. You learn as much about the waterfall when you scoop up a few inert H2O molecules as you learn about how healthy a person is by sequencing their genome: close to nothing.
The waterfall cannot be understood from its parts, only from its movement. And once the flow stops, there is no more waterfall. The waterfall is not a thing that appears and disappears. It is a process—a process that flows and stops flowing. Like a waterfall, you are not a thing. You are a process—an energetic process, to be precise.
Your fundamentally energetic nature has two main implications. The first is that as a dynamic process, you are bound to change. Your body continually sheds, kills and makes cells. Your mind also changes. Some parts of your mind, such as your personality, are relatively stable. But then again, that can change, too—when you are “hangry,” for instance, and become less than your best self. That’s an energy deficit changing your mind.
Some drugs can dramatically change your mind. Psychedelics, for example, act on the serotonin system to desynchronize the human brain. They also dissolve the sense of self, or “ego.” Change brain-energy patterns, change the mind. The mind, then, may essentially be an energy pattern. Further, energy flowing through your brain somehow feels like something. As Nirosha Murugan of Wilfrid Laurier University in Ontario and I recently postulated in a paper, humans may be wired to experience excessive resistance to energy flow as aversive. In contrast, smoothly flowing energy, as occurs after an enjoyable exercise session or when you are working on a stimulating project, feels good. When energy ceases flowing to your brain—if your heart stops, for example—your conscious awareness quickly fades, and you no longer are.
Does all this say anything useful about my original questions? I suspect we now have answers. The key to life and health may lie in how easily energy flows through your mitochondria with each breath you take. So next time you skip that appealing sugary treat, go outside for a stroll, hit the gym or decide to spend time with someone you care about, know that you are supporting your mitochondria. Keeping energy flowing through your mitochondrial collective may be the key to good health and a meaningful life.